By agreement with the publisher, this book is accessible by the search feature, but cannot be browsed.
NCBI Bookshelf. A service of the National Library of Medicine, National Institutes of Health.
Siegel GJ, Agranoff BW, Albers RW, et al., editors. Basic Neurochemistry: Molecular, Cellular and Medical Aspects. 6th edition. Philadelphia: Lippincott-Raven; 1999.

Basic Neurochemistry: Molecular, Cellular and Medical Aspects. 6th edition.
Show detailsThe myelin sheath is a greatly extended and modified plasma membrane wrapped around the nerve axon in a spiral fashion [1]. The myelin membranes originate from and are a part of the Schwann cells in the peripheral nervous system (PNS) and the oligodendroglial cells in the central nervous system (CNS) (see Chap. 1). Each myelin-generating cell furnishes myelin for only one segment of any given axon. The periodic interruptions where short portions of the axon are left uncovered by myelin are the nodes of Ranvier, and they are critical to the functioning of myelin.
Myelin facilitates conduction
Myelin is an electrical insulator; however, its function of facilitating conduction in axons has no exact analogy in electrical circuitry. In unmyelinated fibers, impulse conduction is propagated by local circuits of ion current that flow into the active region of the axonal membrane, through the axon and out through adjacent sections of the membrane (Fig. 4-1). These local circuits depolarize the adjacent piece of membrane in a continuous, sequential fashion. In myelinated axons, the excitable axonal membrane is exposed to the extracellular space only at the nodes of Ranvier; this is the location of sodium channels [2]. When the membrane at the node is excited, the local circuit generated cannot flow through the high-resistance sheath and, therefore, flows out through and depolarizes the membrane at the next node, which might be 1 mm or farther away (Fig. 4-1). The low capacitance of the sheath means that little energy is required to depolarize the remaining membrane between the nodes, which results in local circuit spreading at an increased speed. Active excitation of the axonal membrane jumps from node to node; this form of impulse propagation is called saltatory conduction (Latin saltare, “to jump”). Such movement of the wave of depolarization is much more rapid than in unmyelinated fibers. Furthermore, because only the nodes of Ranvier are excited during conduction in myelinated fibers, Na+ flux into the nerve is much less than in unmyelinated fibers, where the entire membrane is involved. An example of the advantage of myelination is obtained by comparison of two different nerve fibers, both of which conduct at 25 m/sec at 20°C. The 500-mm diameter unmyelinated giant axon of the squid requires 5,000 times as much energy and occupies about 1,500 times as much space as the 12-mm diameter myelinated nerve in the frog.
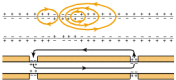
Figure 4-1
Impulse conduction in unmyelinated (top) and myelinated (bottom) fibers. Arrows show the flow of action currents in local circuits into the active region of the membrane. In unmyelinated fibers, the circuits flow through the adjacent piece of membrane, (more...)
Conduction velocity in myelinated fibers is proportional to the diameter, while in unmyelinated fibers it is proportional to the square root of the diameter. Thus, differences in energy and space requirements between the two types of fiber are exaggerated at higher conduction velocities. If nerves were not myelinated and equivalent conduction velocities were maintained, the human spinal cord would need to be as large as a good-sized tree trunk. Myelin, then, facilitates conduction while conserving space and energy [3].
Myelin has a characteristic ultrastructure
Myelin, as well as many of its morphological features, such as nodes of Ranvier and Schmidt-Lantermann clefts, can be seen readily with light microscopy (Fig. 4-2). Further insight comes from biophysical studies of structures with parallel axons: sciatic nerve as representative of the PNS and optic nerve or tract as representative of the CNS. Myelin, when examined by polarized light, exhibits both a lipid-dependent and a protein-dependent birefringence. Low-angle X-ray diffraction studies of myelin provide electron-density plots of the repeating unit that show three peaks which correspond to protein plus lipid polar groups and two troughs which correspond to lipid hydrocarbon chains. The repeat distance varies somewhat depending on the species and whether the sample is from the CNS or the PNS. Thus, the results from these two techniques are consistent with a protein—lipid—protein—lipid—protein structure, in which the lipid portion is a bimolecular leaflet and adjacent protein layers are different in some way. Data for mammalian optic nerve show a repeat distance of 80 Å (Fig. 4-3). This spacing can accommodate one bimolecular layer of lipid (about 50 Å) and two protein layers (about 15 Å each). The main repeating unit of two such fused unit membranes is twice this figure, or 160 Å [5]. Although it is useful to think of myelin in terms of alternating protein and lipid layers, this concept has been modified somewhat to be compatible with the “fluid mosaic” model of membrane structure, which includes intrinsic transmembrane proteins as well as extrinsic proteins.

Figure 4-2
Light micrograph of a 1-μm Epon section of rabbit peripheral nerve (anterior root) stained with toluidine blue. The myelin sheath appears as a thick black ring around the pale axon. ×600, before 30% reduction. (Courtesy of Dr. Cedric Raine.) (more...)
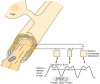
Figure 4-3
A composite diagram summarizing some of the ultrastructural data on CNS myelin. At the top, an oligodendroglial cell is shown connected to the sheath by a process. The cutaway view of the myelin and axon illustrates the relationship of these two structures (more...)
Information concerning myelin structure is also available from electron-microscopic studies, which visualize myelin as a series of protein layers appearing as alternating dark and less dark lines separated by lipid hydrocarbon chains which appear as unstained zones (Figs. 4-4–4-7). There is asymmetry in the staining of the protein layers. The less dark, or intraperiod, line represents the closely apposed outer protein coats of the original cell membrane; the membranes are not actually fused since they can be resolved as a double line at high resolution (Figs. 4-6 and 4-7). The dark, or major period, line is the fused, inner protein coat of the cell membrane. The repeat distances observed by electron microscopy are less than those calculated from the low-angle X-ray diffraction data, a consequence of the considerable shrinkage that takes place after fixation and dehydration. However, the difference in periodicity between PNS and CNS myelin is maintained; peripheral myelin has an average repeat distance of 119 Å and central myelin, 107 Å.
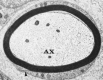
Figure 4-4
Electron micrograph of a single peripheral nerve fiber from rabbit. Note that the myelin sheath has a lamellated structure and is surrounded by Schwann cell cytoplasm. The outer mesaxon (arrowhead) can be seen at the lower left. AX, axon. ×18,000. (more...)

Figure 4-7
A typical CNS myelinated fiber from the spinal cord of an adult dog. Contrast this figure with the PNS fiber in Figure 4-3. The course of the flattened oligodendrocytic process, beginning at the outer tongue (arrow), can be traced. Note that the fiber (more...)
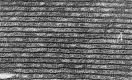
Figure 4-6
Magnification of the myelin sheath of Figure 4-4. Note that the intraperiod line (arrows) at this high resolution is a double structure. ×350,000. (Courtesy of Dr. Cedric Raine.)

Figure 4-5
Higher magnification of Figure 4-4 to show the Schwann cell cytoplasm covered by basal lamina (arrows). ×50,000.
Nodes of Ranvier. Two adjacent segments of myelin on one axon are separated by a node of Ranvier. In this region, the axon is not covered by myelin. At the paranodal region and the Schmidt-Lantermann clefts, the cytoplasmic surfaces of myelin are not compacted and Schwann or glial cell cytoplasm is included within the sheath. To visualize these structures, one may refer to Figures 4-8 and 4-9, which show that if myelin were unrolled from the axon, it would be a flat, spade-shaped sheet surrounded by a tube of cytoplasm. Thus, as shown in electron micrographs of longitudinal sections of axon paranodal regions, the major dense line formed by apposition of the cytoplasmic faces opens up at the edges of the sheet, enclosing cytoplasm within a loop (see Figs. 4-3 and 4-9). These loop-shaped terminations of the sheath at the node are called lateral loops. The loops form membrane complexes with the axolemma called transverse bands, whereas myelin in the internodal region is separated from the axon by a gap of periaxonal space. The transverse bands are helical structures that seal the myelin to the axolemma but provide, by spaces between them, a tortuous path from the extracellular space to the periaxonal space.
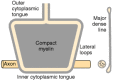
Figure 4-8
A diagram showing the appearance of CNS myelin if it were unrolled from the axon. One can visualize this structure arising from Figure 4-3 if the glial cell process were pulled straight up and the myelin layers separated at the intermediate period line. (more...)

Figure 4-9
A diagram similar to Figure 4-8 but showing one Schwann cell and its myelin sheath unrolled from a peripheral axon. The sheet of PNS myelin is, like CNS myelin, surrounded by a tube of cytoplasm and has additional tubes of cytoplasm, which make up the (more...)
Schmidt-Lantermann clefts are structures where the cytoplasmic surfaces of the myelin sheath have not compacted to form the major dense line and, instead, contain Schwann or glial cell cytoplasm (Fig. 4-9). These regions are common in peripheral myelinated axons but rare in the CNS. These inclusions of cytoplasm are present in each layer of myelin. The clefts can be visualized in the unrolled myelin sheet as tubes of cytoplasm similar to the tubes making up the lateral loops but in the middle regions of the sheet, rather than at the edges (Fig. 4-9).
Myelin is an extension of a cell membrane
In the PNS, myelination is preceded by invasion of the nerve bundle by Schwann cells, rapid multiplication of these cells and segregation of the individual axons by Schwann cell processes. Smaller axons (≤1 μm), which will remain unmyelinated, are segregated; several may be enclosed in one cell, each within its own pocket, similar to the structure shown in Figure 4-10A. Large axons (≥1 μm) destined for myelination are enclosed singly, one cell per axon per internode. These cells line up along the axons with intervals between them; the intervals become the nodes of Ranvier.
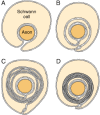
Figure 4-10
Myelin formation in the peripheral nervous system. A: The Schwann cell has surrounded the axon, but the external surfaces of the plasma membrane have not yet fused in the mesaxon. B: The mesaxon has fused into a five-layered structure and spiraled once (more...)
Before myelination, the axon lies in an invagination of the Schwann cell (Fig. 4-10A). The plasmalemma of the cell then surrounds the axon and joins to form a double-membrane structure that communicates with the cell surface. This structure, called the mesaxon, elongates around the axon in a spiral fashion (Fig. 4-10). Thus, formation of myelin topologically resembles rolling up a sleeping bag; the mesaxon winds about the axon, and the cytoplasmic surfaces condense into a compact myelin sheath and form the major dense line. The two external surfaces form the myelin intraperiod line.
In the CNS, the structures of myelin are formed by the oligodendroglial cell [7]. This has many similarities but also points of difference with respect to myelination in the PNS. CNS nerve fibers are not separated by connective tissue, nor are they surrounded by cell cytoplasm, and specific glial nuclei are not obviously associated with particular myelinated fibers. CNS myelin is a spiral structure similar to PNS myelin; it has an inner mesaxon and an outer mesaxon that ends in a loop, or tongue, of glial cytoplasm (Fig. 4-3). Unlike the peripheral nerve, where the sheath is surrounded by Schwann cell cytoplasm, the cytoplasmic tongue in the CNS is restricted to a small portion of the sheath. This glial tongue is continuous with the plasma membrane of the oligodendroglial cell through slender processes. One glial cell can myelinate 40 or more separate axons [8].
Myelin deposition in the PNS may result in a single axon having up to 100 myelin layers; therefore, it is improbable that myelin is laid down by a simple rotation of the Schwann cell nucleus around the axon. In the CNS, such a postulate is precluded by the fact that one glial cell can myelinate several axons. During myelination, there are increases in the length of the internode, the diameter of the axon and the number of myelin layers. Myelin, therefore, expands in all planes at once. Any mechanism to account for this growth must assume that the membrane system is able to expand and contract and that layers slip over each other.
Myelin can be isolated in high yield and purity by conventional methods of subcellular fractionation
If CNS tissue is homogenized in media of low ionic strength, myelin peels off the axons and reforms in vesicles of the size range of nuclei and mitochondria. Because of their high lipid content, these myelin vesicles have the lowest intrinsic density of any membrane fraction of the nervous system. Procedures for isolation of myelin take advantage of both the large vesicle size and the low density [9].
In a widely used method, a homogenate of rodent nervous tissue, or dissected white matter in the case of larger animals, in isotonic sucrose (0.3 M) is layered directly onto 0.85 M sucrose and centrifuged at high speed. Mitochondria and synaptosomes sediment through the denser sucrose, and many of the smaller membrane fragments from other organelles remain in the 0.3 M sucrose layer. A crude myelin layer collects at the interface. The major impurities, microsomes and axoplasm trapped in the vesicles during the homogenization procedure are released by subjecting the myelin to osmotic shock in distilled water. The larger myelin particles can then be separated from the smaller, membranous material by low-speed centrifugation or by repeating the density gradient centrifugation on continuous or discontinuous gradients, usually of sucrose. Preparations of purified myelin can be subdivided further and arbitrarily into fractions of different densities by centrifugation on expanded continuous or discontinuous density gradients. These fractions differ somewhat in composition.
Demonstration of purity for a myelin preparation includes electron-microscopic appearance; however, the difficulty of identifying small membrane vesicles of microsomes in a field of myelin membranes and the well-known sampling problems inherent in electron microscopy make this characterization unreliable after a certain purity level has been reached.
Markers characteristic of myelin include certain proteins, lipids and enzymes described in the following sections. Although such assays are useful, like electron microscopy they are not sensitive to small amounts of impurities. If purity of a myelin preparation is an issue, it is important to assay contamination of myelin by other subcellular fractions using markers such as succinic dehydrogenase for mitochondria; Na,K-ATPase and 5′-nucleotidase for plasma membranes; NADH-cytochrome-C reductase for microsomes; DNA for nuclei; RNA for nuclei, ribosomes and microsomes; lactate dehydrogenase for cytosol; β-glucosidase for lysosomes; and acetylcholinesterase for neuronal fragments. Although all of these markers are low in purified myelin and set an outside limit for levels of contamination by other membranes, the actual contamination may be less than calculated by such methods since low levels of many different enzymes appear to be intrinsic to myelin.
Peripheral nerve myelin can be isolated by similar techniques, but especially vigorous homogenization conditions are required because of the large amounts of connective tissue and, sometimes, adipose tissue present in the nerve. The slightly lesser density of PNS myelin requires some adjustment of gradient composition to prevent loss of myelin.
- The Myelin Sheath - Basic NeurochemistryThe Myelin Sheath - Basic Neurochemistry
- Epidermolysis Bullosa Simplex - GeneReviews®Epidermolysis Bullosa Simplex - GeneReviews®
- PP_RS28050 [Pseudomonas putida KT2440]PP_RS28050 [Pseudomonas putida KT2440]Gene ID:1043341Gene
- Rad21l RAD21-like (S. pombe) [Mus musculus]Rad21l RAD21-like (S. pombe) [Mus musculus]Gene ID:668929Gene
- RGSL1 regulator of G protein signaling like 1 [Homo sapiens]RGSL1 regulator of G protein signaling like 1 [Homo sapiens]Gene ID:353299Gene
Your browsing activity is empty.
Activity recording is turned off.
See more...