By agreement with the publisher, this book is accessible by the search feature, but cannot be browsed.
NCBI Bookshelf. A service of the National Library of Medicine, National Institutes of Health.
Alberts B, Johnson A, Lewis J, et al. Molecular Biology of the Cell. 4th edition. New York: Garland Science; 2002.

Molecular Biology of the Cell. 4th edition.
Show detailsMost cells of the animal body are motile, and in the developing embryo their movements are often extensive, dramatic, and surprising. Controlled changes of gene expression create ordered arrays of cells in different states; cell movements rearrange these cellular building blocks and put them in their proper places. The genes that the cells express determine how they move; in this sense, the control of gene expression is the primary phenomenon. But the cell movements are also crucial, and no less in need of explanation if we want to understand how the architecture of the body is created. In this section, we examine this topic in the context of vertebrate development. We take as our main example the frog Xenopus laevis (Figure 21-65), where cell movements have been well studied, though we shall also draw on evidence from chick, zebrafish and mouse.
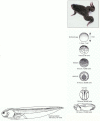
Figure 21-65
Synopsis of the development of Xenopus laevis from newly fertilized egg to feeding tadpole. The adult frog is shown in the photograph at the top. The developmental stages are viewed from the side, except for the 10-hour and 19-hour embryos, which are (more...)
The Polarity of the Amphibian Embryo Depends on the Polarity of the Egg
The Xenopus egg is a large cell, just over a millimeter in diameter (Figure 21-66A). The light-colored lower end of the egg is called the vegetal pole; the dark-colored upper end is called the animal pole. The animal and vegetal hemispheres contain different selections of mRNA molecules and other cell components, which become allocated to separate cells as the egg cell divides after fertilization. Near the vegetal pole, for example, there is an accumulation of mRNAs coding for the gene regulatory protein VegT (a DNA-binding protein of the T-box family) and for signal proteins of the TGFβ superfamily, as well as some ready-made protein components of the Wnt signaling pathway (Figure 21-66B). As a result, the cells that inherit vegetal cytoplasm will produce signals to organize the behavior of adjacent cells and are committed to form the gut—the innermost tissue of the body; the cells that inherit animal cytoplasm will form the outer tissues.
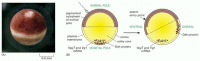
Figure 21-66
The Xenopus egg and its asymmetries. (A) Side view of an egg photographed just before fertilization. (B) The asymmetric distribution of molecules inside the egg, and how this changes following fertilization so as to define a dorsoventral as well as an (more...)
Fertilization initiates a complex series of movements that will eventually tuck the vegetal cells and cells from the equatorial (middle) region of the animal-vegetal axis into the interior. In the process, the three principal axes of the body are established: anteroposterior, from head to tail; dorsoventral, from back to belly; and mediolateral, from the midline outward to the left or to the right. The animal-vegetal asymmetry of the unfertilized egg is sufficient to define only one of these future body axes—the anteroposterior. Fertilization triggers an intracellular movement that gives the egg an additional asymmetry defining a dorsoventral difference. Following entry of the sperm, the outer, actin-rich cortex of the egg cytoplasm rotates relative to the central core of the egg, so that the animal pole of the cortex is slightly shifted to the future ventral side. Treatments that block the rotation allow cleavage to occur normally but produce an embryo with a central gut and no dorsal structures or dorsoventral asymmetry.
The direction of the cortical rotation is biased according to the point of sperm entry, perhaps through the centrosome that the sperm brings into the egg, and the movement is associated with a reorganization of microtubules in the egg cytoplasm. This leads to a microtubule-based transport of the protein Dishevelled, a downstream component of the Wnt signaling pathway, toward the future dorsal side (see Figure 21-66B). The subcellular region in which Dishevelled thus becomes concentrated gives rise to cells that behave as though they have received a Wnt signal and express a dorsal-specific set of genes as a result. These cells will generate further signals to organize the dorsoventral axis of the body.
Cleavage Produces Many Cells from One
The cortical rotation is completed in about an hour after fertilization and is followed by cleavage, in which the single large egg cell rapidly subdivides by repeated mitosis into many smaller cells, or blastomeres, without any change in total mass (Figure 21-67). In this way, the determinants distributed asymmetrically in the egg become partitioned into separate cells, with different fates (Figure 21-68).

Figure 21-67
The stages of cleavage in Xenopus. The cleavage divisions rapidly subdivide the egg into many smaller cells. All the cells divide synchronously for the first 12 cleavages, but the divisions are asymmetric, so that the lower, vegetal cells, encumbered (more...)
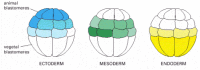
Figure 21-68
The origins of the three germ layers can be traced back to distinct blastomeres of the embryo in its early cleavage stages. The endoderm derives from the most vegetal blastomeres, the ectoderm from the most animal, and the mesoderm from a middle set that (more...)
These first cell divisions in Xenopus have a cycle time of about 30 minutes, with a direct alternation of S and M phases, as discussed in Chapter 17. The very high rate of DNA replication and mitosis seems to preclude gene transcription (although protein synthesis occurs), and the cleaving embryo is almost entirely dependent on reserves of RNA, protein, membrane, and other materials that accumulated in the egg while it developed as an oocyte in the mother. After about 12 cycles of cleavage (7 hours), the cell division rate slows down, the cell cycles begin to follow the standard pattern with G1 and G2 phases intervening between the S and M phases, and transcription of the embryo's genome begins. This event is called the mid-blastula transition.
Gastrulation Transforms a Hollow Ball of Cells into a Three-Layered Structure with a Primitive Gut
During the period of cleavage, the frog embryo becomes transformed from a solid sphere of cells into something more like a hollow ball, with an internal fluid-filled cavity surrounded by cells that cohere to form an epithelial sheet. The embryo is now termed a blastula (Figure 21-69).
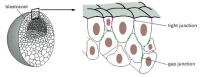
Figure 21-69
The blastula. In the outermost regions of the embryo, tight junctions between the blastomeres begin to create an epithelial sheet that isolates the interior of the embryo from the external medium. Na+ is pumped across this sheet into the spaces in the (more...)
Soon after this, the coordinated movements of gastrulation begin. This dramatic process transforms the simple hollow ball of cells into a multilayered structure with a central gut tube and bilateral symmetry: by a more elaborate version of the process outlined earlier for the sea urchin (see Figure 21-3), many of the cells on the outside of the embryo are moved inside it. Subsequent development depends on the interactions of the inner, outer, and middle layers of cells thus formed: the endoderm on the inside, consisting of the cells that have moved into the interior to form the primitive gut; the ectoderm on the outside, consisting of cells that have remained external; and the mesoderm between them, consisting of cells that detach from the epithelium to form a more loosely organized embryonic connective tissue (Figure 21-70). From these three germ layers, the tissues of the adult vertebrate body will be generated, preserving the basic body plan established through gastrulation.
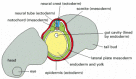
Figure 21-70
A cross section through the trunk of an amphibian embryo after the end of gastrulation, showing the arrangement of endodermal, mesodermal and ectodermal tissues. The endoderm will form the epithelial lining of the gut, from the mouth to the anus. It gives (more...)
The Movements of Gastrulation Are Precisely Predictable
The pattern of gastrulation movements that creates the germ layers and establishes the body axes is described for Xenopus in Figure 21-71. The details are complex, but the principles are simple.
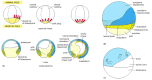
Figure 21-71
Gastrulation in Xenopus. (A) The external views (above) show the embryo as a semitransparent object, seen from the side; with the directions of cell movement indicated by red arrows, cross sections (below) are cut in the median plane (the plane of the (more...)
Cells of the future endoderm are folded into the interior, or involuted, in succession. The cells that were near the vegetal pole of the blastula involute first, turning inward and then moving up toward the animal pole to form the most anterior part of the gut. As they near the animal pole, these leading endoderm cells will signal to the overlying ectoderm to define the anterior extremity of the head. The mouth will eventually develop as a hole formed at an anterior site where endoderm and ectoderm come into direct contact. Meanwhile, future mesoderm cells, destined to detach from the epithelial sheet to form the sandwich filling between endoderm and ectoderm, tuck into the interior along with the endoderm cells, and also move up toward the animal pole. The cells that are first to involute go to form parts of the head, and those that are last form parts of the tail. In this way, the anteroposterior axis of the final embryo is laid down sequentially.
The anteroposterior movements go hand in hand with movements that organize the dorsoventral axis of the body. Gastrulation begins on the side of the blastula that has been marked out as dorsal by the cortical rotation. Here, involution of cells into the interior starts with a short indentation that rapidly extends to form the blastopore—a line of invagination that curves around to encircle the vegetal pole. The site where the invagination starts defines the dorsal lip of the blastopore. As we shall see, this tissue plays a leading part in subsequent events and gives rise to the central dorsal structures of the main body axis.
Chemical Signals Trigger the Mechanical Processes
The movements of gastrulation are triggered by chemical signals from the vegetal blastomeres. Several proteins of the TGFβ superfamily are secreted by these cells and act on the blastomeres above them. If these signals are blocked, gastrulation is disrupted and no mesodermal cell types are generated. The local activation of components of the Wnt signaling pathway on the dorsal side of the embryo (as a result of the earlier cortical rotation, see Figure 21-66) modifies the action of the other signals so as to induce development of the special cells that form the dorsal lip of the blastopore (Figure 21-72).
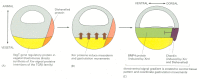
Figure 21-72
A current view of the main inductive signals organizing the events of gastrulation. (A) The distribution of axis-determining molecules in the blastula results from inheritance of different parts of the cytoplasm of the fertilized frog egg. The VegT gene (more...)
The dorsal lip of the blastopore plays a central role in gastrulation not just in a geometrical sense, but as a powerful new source of control. If the dorsal lip of the blastopore is excised from an embryo at the beginning of gastrulation and grafted into another embryo but in a different position, the host embryo initiates gastrulation both at the site of its own dorsal lip and at the site of the graft. The movements of gastrulation at the second site entail the formation of a second whole set of body structures, and a double embryo (Siamese twins) results (see Figure 21-6B).
Evidently, the dorsal lip of the blastopore is the source of a signal (or signals) coordinating both the movements of gastrulation and the pattern of specialization of the tissues in its neighborhood. Because of this crucial role in organizing the formation of the main body axis, the dorsal lip of the blastopore is known as the Organizer (or Spemann's Organizer, after its co-discoverer). It is the oldest and most famous example of an embryonic signaling center.
Active Changes of Cell Packing Provide a Driving Force for Gastrulation
The Organizer controls the dorsoventral pattern of cell differentiation in its neighborhood by secreting proteins, including chordin that inhibit the action of the TGFβ-like signals (specifically BMP proteins) produced by the more ventral cells (Figure 21-72C). This sets up a gradient of signaling activity—a morphogen gradient, whose local value tells cells how far they lie from the Organizer (see Figure 21-14). But how is the pattern of cell movements organized in mechanical terms, and what are the forces that bring it about?
Gastrulation begins with changes in the shape of the cells at the site of the blastopore. In the amphibian these are called bottle cells: they have broad bodies and narrow necks that anchor them to the surface of the epithelium (Figure 21-73), and they may help to force the epithelium to curve and so to tuck inward, producing the initial indentation seen from outside. Once this first tuck has formed, cells can continue to pass into the interior as a sheet to form the gut and mesoderm. The movement seems to be driven mainly by an active repacking of the cells, especially those in the involuting regions around the Organizer (see Figure 21-73). Here convergent extension occurs. Small square fragments of tissue from these regions, isolated in culture, will spontaneously narrow and elongate through a rearrangement of the cells, just as they would in the embryo in the process of converging toward the dorsal midline, turning inward around the blastopore lip, and then elongating to form the main axis of the body.
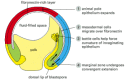
Figure 21-73
Cell movements in gastrulation. A section through a gastrulating Xenopus embryo, cut in the same plane as in Figure 21-71, indicating the four main types of movement that gastrulation involves. The animal pole epithelium expands by cell rearrangement, (more...)
To bring about this remarkable transformation, the individual cells have to crawl over one another in a coordinated way (Figure 21-74). The alignment of their movements appears to depend on the same machinery we encountered in the worm and the fly controlling planar cell polarity: the Frizzled/Dishevelled polarity-signaling pathway. When this pathway is blocked—for example, by a dominant-negative form of Dishevelled—convergent extension fails to occur.
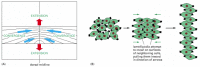
Figure 21-74
Convergent extension and its cellular basis. (A) The pattern of convergent extension in the marginal zone of a gastrula as viewed from the dorsal aspect. Blue arrows represent convergence toward the dorsal midline, red arrows represent extension of the (more...)
Changing Patterns of Cell Adhesion Molecules Force Cells Into New Arrangements
Patterns of gene expression govern embryonic cell movements in many different ways. They regulate cell motility, cell shape, and the production of signals for guidance. Very importantly, they also determine the sets of adhesion molecules that the cells display on their surfaces. Through changes in its surface molecules, a cell can break old attachments and make new ones. Cells in one region may develop surface properties that make them cohere with one another and become segregated from a neighboring group of cells whose surface chemistry is different.
Experiments done half a century ago on early amphibian embryos showed that the effects of selective cell-cell adhesion can be so powerful that they can bring about an approximate reconstruction of the normal structure of an early postgastrulation embryo even after the cells have been artificially dissociated. When these cells are reaggregated into a random mixture, the cells sort out spontaneously according to their original characters (Figure 21-75). As discussed in Chapter 19, a central role in such phenomena is played by the cadherins—a large and varied family of evolutionarily related Ca2+-dependent cell-cell adhesion proteins. These and other cell-cell-adhesion molecules are differentially expressed in the various tissues of the early embryo, and antibodies against them interfere with the normal selective adhesion between cells of a similar type.
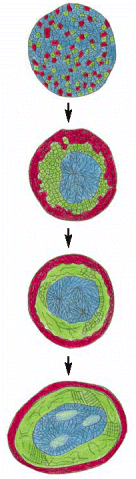
Figure 21-75
Sorting out. Cells from different parts of an early amphibian embryo will sort out according to their origins. In the classical experiment shown here, mesoderm cells (green), neural plate cells (blue), and epidermal cells (red) have been disaggregated (more...)
Changes in the patterns of expression of the various cadherins correlate closely with the changing patterns of association among cells during gastrulation, neurulation, and somite formation (see Figure 19-25). These rearrangements are likely to be regulated and driven in part by the cadherin pattern. In particular, cadherins appear to have a major role in controlling the formation and dissolution of epithelial sheets and clusters of cells. They not only glue one cell to another, but also provide anchorage for intracellular actin filaments at the sites of cell-cell adhesion. In this way the pattern of stresses and movements in the developing tissue is regulated according to the pattern of adhesions.
The Notochord Elongates, While the Neural Plate Rolls Up to Form the Neural Tube
Gastrulation is only the first—though perhaps the most dramatic—of a dizzying variety of cell movements that shape the parts of the body. We have space to discuss only a few of these.
In the embryo just after gastrulation, the layer of mesoderm is divided into separate slabs on the left and right sides of the body. Defining the central body axis, and effecting this separation, is the very early specialization of the mesoderm known as the notochord. This slender rod of cells, with ectoderm above it, endoderm below it, and mesoderm on either side (see Figure 21-70), derives from the cells of the Organizer itself. The notochordal cells are characterized by expression of a gene regulatory protein called Brachyury (Greek for “short-tail”, from the mutant phenotype); this belongs to the same T-box family as the VegT protein in the vegetal blastomeres.
As the notochordal cells pass around the dorsal lip of the blastopore and move into the interior of the embryo, they form a column of tissue that elongates dramatically by convergent extension. The cells of the notochord also become swollen with vacuoles, so that the rod elongates still further and stretches out the embryo. The notochord is the defining peculiarity of the chordates—the phylum to which the vertebrates belong. It is one of the major vertebrate features that do not have any apparent counterpart in Drosophila. In the most primitive chordates, which have no vertebrae, the notochord persists as a primitive substitute for a vertebral column. In vertebrates it serves as a core around which other mesodermal cells will eventually gather to form the vertebrae.
In the overlying sheet of ectoderm, meanwhile, other movements are occurring to form the rudiments of the nervous system. In a process known as neurulation, a broad central region of ectoderm, called the neural plate, thickens, rolls up into a tube, and pinches off from the rest of the cell sheet. The tube thus created from the ectoderm is called the neural tube; it will form the brain and the spinal cord (Figure 21-76).
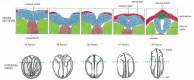
Figure 21-76
Neural tube formation in Xenopus. The external views are from the dorsal aspect. The cross sections are cut in a plane indicated by the broken lines. (After T.E. Schroeder, J. Embryol. Exp. Morphol. 23:427–462, 1970. © The Company of Biologists.) (more...)
The mechanics of neurulation depend on changes of cell packing and cell shape that make the epithelium roll up into a tube (Figure 21-77). Signals initially from the Organizer and later from the underlying notochord and mesoderm define the extent of the neural plate, induce the movements that make it roll up, and help to organize the internal pattern of the neural tube. The notochord in particular secretes Sonic hedgehog protein—a homolog of the Drosophila signal protein Hedgehog—and this acts as a morphogen to control gene expression in the neighboring tissues (see Figure 21-94).
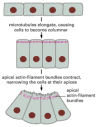
Figure 21-77
The bending of an epithelium through cell shape changes mediated by microtubules and actin filaments. The diagram is based on observations of neurulation in newts and salamanders, where the epithelium is only one cell layer thick. As the apical ends of (more...)
A Gene-Expression Oscillator Controls Segmentation of the Mesoderm Into Somites
Genetically regulated changes in cell adhesion underlie one of the most striking and characteristic processes in vertebrate development—the formation of the segments of the body axis.
On either side of the newly formed neural tube lies a slab of mesoderm (see Figure 21-70). To form the repetitive series of vertebrae, ribs, and segmental muscles, this slab breaks up into separate blocks, or somites—cohesive groups of cells, separated by clefts. Figure 21-78A shows the process as it occurs in the chick embryo. The somites form one after another, starting in the head and ending in the tail. Depending on the species, the final number of somites ranges from less than 50 (in a frog or a bird) to more than 300 (in a snake). The posterior, most immature part of the mesodermal slab, called the presomitic mesoderm, supplies the necessary tissue: as it retreats tailward, extending the embryo, it deposits a trail of somites.
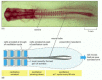
Figure 21-78
Somite formation in the chick embryo. (A) A chick embryo at 40 hours of incubation. (B) How the temporal oscillation of c-hairy-1 expression in the presomitic mesoderm becomes converted into a spatial pattern of gene expression in the formed somites. (more...)
Formation of the cleft between one somite and the next is foreshadowed by an alternating spatial pattern of gene expression in the presomitic mesoderm: cells about to form the posterior part of a new somite switch on expression of one set of genes, while those destined to form the anterior part of the next somite switch on expression of another set. The genes that mark out the somite pattern in this way include at least one member of the cadherin superfamily (called paraxial protocadherin), and the intersomitic cleft forms where cells that express it confront cells that do not. Selective cohesion resulting from differential gene expression (either of this gene or some other) thus seems to be the underlying cause of the physical segmentation observed.
The problem then is to understand how the repetitive alternating pattern of gene expression is set up. Studies in the chick embryo have provided an answer. In the posterior part of the presomitic mesoderm, expression of certain genes—in particular the gene c-hairy-1, a homolog of the Drosophila pair-rule gene hairy—is found to oscillate in time. The length of one complete oscillation cycle of this gene-expression “clock” (90 minutes in the chick) equals the time taken to lay down one further somite. As cells mature and emerge from the presomitic mesoderm to form somites, their oscillation is arrested. c-hairy-1 encodes a gene regulatory protein, and the cells arrested at the peak of their c-hairy-1 oscillation cycle express one set of genes, while those arrested at the trough of the cycle express another (Figure 21-78B). In this way, the temporal oscillation of gene expression in the presomitic mesoderm leaves its trace in a spatially periodic pattern of gene expression in the maturing mesoderm, and this in turn dictates how the tissue will break up into physically separate blocks. The mechanism that generates the temporal oscillation itself is unknown.
Most of the cells of each newly formed somite will rapidly differentiate to form a block of muscle, corresponding to one muscle segment of the main body axis. The embryo can (and does) now begin to wriggle. Separate subsets of the somite cells will go to form the vertebrae and other connective tissues such as dermis. A further subset detach from the somite and migrate away into the lateral unsegmented mesoderm, crawling through the spaces between other cells: these emigrants will give rise to almost all the other skeletal muscle cells in the body, including those of the limbs.
Embryonic Tissues Are Invaded in a Strictly Controlled Fashion by Migratory Cells
The muscle-cell precursors, or myoblasts, that emigrate from the somites are determined but not overtly differentiated. In the tissues that they colonize they will mingle with other classes of cells from which they appear practically indistinguishable; but they will maintain expression of myoblast-specific gene regulatory proteins (such as members of the MyoD family), and when the time comes for differentiation, they, and they alone, will turn into muscle cells (Figure 21-79).
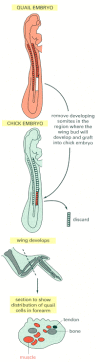
Figure 21-79
The migratory origin of limb muscle cells. The migrations can be traced by grafting cells from a quail embryo into a chick embryo; the two species are very similar in their development, but the quail cells are recognizable by the distinctive appearance (more...)
The eventual pattern of muscles—in the limbs, for example—is determined by the routes that the migrant cells follow and the selection of sites that they colonize. The embryonic connective tissues form the framework through which the myoblasts travel and provide signals that guide their distribution. No matter which somite they come from, myoblasts that migrate into a forelimb bud will form the pattern of muscles appropriate to a forelimb, and those that migrate into a hindlimb bud will form the pattern appropriate to a hindlimb.
Other classes of migrant cells, meanwhile, select different routes for their travels. Along the line where the neural tube pinches off from the future epidermis, a number of ectodermal cells break loose from the epithelium and also migrate as individuals out through the mesoderm (Figure 21-80). These are the cells of the neural crest; they will give rise to almost all of the neurons and glial cells of the peripheral nervous system, as well as the pigment cells of the skin and many connective tissues in the head, including bones of the skull and jaws. Other important migrants are the precursors of the blood cells, of the germ cells, and of many groups of neurons within the central nervous system, as well as the endothelial cells that form blood vessels. Each of these classes of travelers will colonize a different set of sites. As a result of such invasions, most tissues in the vertebrate body are mixtures of cells of different characters derived from widely separate parts of the embryo.
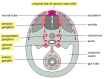
Figure 21-80
The main pathways of neural crest cell migration. A chick embryo is shown in a schematic cross-section through the middle part of the trunk. The cells that take the pathway just beneath the ectoderm will form pigment cells of the skin; those that take (more...)
As a migrant cell travels through the embryonic tissues, it repeatedly extends projections that probe its immediate surroundings, testing for subtle cues to which it is particularly sensitive by virtue of its specific assortment of cell-surface receptor proteins. Inside the cell these receptor proteins are connected to the cytoskeleton, which moves the cell along. Some extracellular matrix materials, such as the protein fibronectin, provide adhesive sites that help the cell to advance; others, such as chondroitin sulfate proteoglycan, inhibit locomotion and repel immigration. The nonmigrant cells along the pathway may likewise have inviting or repellent surfaces, or may even extend filopodia that touch the migrant cell and affect its behavior. An incessant tug-of-war between opposing tentative attachments made by the migrant cell leads to a net movement in the most favored direction until the cell finds a site where it can form a lasting attachment. Other factors such as chemotaxis and interactions among the migratory cells may also contribute to their guidance.
The Distribution of Migrant Cells Depends on Survival Factors as Well as Guidance Cues
The final distribution of migrant cells depends not only on the routes they take, but also on whether they survive the journey and thrive in the environment they find at the journey's end. Specific sites provide survival factors needed by specific types of migrant. For example, the neural crest cells that give rise to the pigment cells of the skin and the nerve cells of the gut depend on a peptide factor called Endothelin-3 that is secreted by tissues on the migration pathways; mutant mice and humans defective in the gene for this factor or its receptor have non-pigmented (albino) patches and potentially lethal gut malformations resulting from the lack of gut innervation (a condition called megacolon, because the colon becomes hugely distended).
Germ cells, blood cell precursors, and neural-crest-derived pigment cells all appear to share at least one common requirement for survival. This involves a transmembrane receptor, called the Kit protein, in the membrane of the migrant cells and a ligand, called the Steel factor, produced by the cells of the tissue through which the cells migrate and/or in which they come to settle. Individuals with mutations in the genes for either of these proteins are deficient in their pigmentation, their supply of blood cells, and their production of germ cells (Figure 21-81).
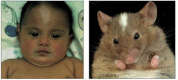
Figure 21-81
Effect of mutations in the kit gene. Both the baby and the mouse are heterozygous for a loss-of-function mutation that leaves them with only half the normal quantity of kit gene product. In both cases pigmentation is defective because pigment cells depend (more...)
Left-Right Asymmetry of the Vertebrate Body Derives From Molecular Asymmetry in the Early Embryo
Vertebrates may look bilaterally symmetrical from the outside, but many of their internal organs—the heart, the stomach, the liver, and so on—are highly asymmetric. This asymmetry is quite reproducible: 99.98% of people have their heart on the left. We have seen how a vertebrate embryo develops its internal and external tissue layers and its anteroposterior and dorsoventral axes. But how does the left-right asymmetry arise?
Genetic studies in mammals show that this problem can be broken down into two distinct questions—one concerning the creation of asymmetry and the other concerning its orientation. Several mutations are known, in humans and in mice, that cause a randomization of the left-right axis: 50% of the mutant individuals have their internal organs arranged in the normal way, while the other 50% have an inverted anatomy, with the heart on the right. In these individuals, it seems, the mechanism that makes the left and right sides different has functioned correctly, but the mechanism that decides between the two possible orientations of the left-right axis is defective.
A key to the basis of these phenomena comes from the discovery of molecular asymmetries that precede the first gross anatomical asymmetries. The earliest signs are seen in patterns of gene expression in the neighborhood of the node—the homolog in mouse and chick of the frog Organizer. Several members of the TGFβ superfamily are expressed asymmetrically in this region (not only in the mouse, but also in chick, frog and zebrafish), as are some other signal proteins including Sonic hedgehog (Figure 21-82). These factors regulate one another's production, producing a cascade of effects through which an asymmetry in the immediate neighborhood of the node apparently becomes self-reinforcing and is relayed outward to create an asymmetric pattern of gene expression over a much larger region.
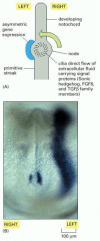
Figure 21-82
Helical beating of cilia at the node, and the origins of left-right asymmetry. (A) The beating of the cilia drives a current towards one side of the node. Various signal proteins are produced in this neighborhood, and the current is thought to sweep them (more...)
Mutations in some of these signaling components can have the effect of obliterating the asymmetry, so as to give a mirror-symmetrical anatomy. For example, mice with a knock-out mutation in the Lefty-1 gene (coding for a Tgfβ-superfamily member) frequently have the right side converted into a mirror image of the left. The mechanism that normally forces the two sides to be different is still not understood. Somehow, cells neighboring the node on opposite sides must become different even if there is very little difference between them at the outset. One possibility is that some sort of lateral inhibition operates: cells on opposite sides of the node may exchange mutually inhibitory signals, and thereby compete with one another to adopt a left-side (or a right-side) character. Or perhaps some small but influential group of cells, originating near the midline, is forced to one side by the formation of other midline structures.
Whatever the mechanism, the outcome of events at the node in a normal animal must be biased so that left-specific genes are regularly expressed on the left side: there has to be a link between the mechanism that creates asymmetry and the mechanism that orients it. A clue to the orienting mechanism first came to light in a Swedish infertility clinic. A small subset of infertile men were found to have sperm that were immotile because of a defect in the dynein molecules needed for beating of cilia and flagella. These men also suffered from chronic bronchitis and sinusitis because the cilia in their respiratory tract were defective. And strikingly, 50% of them had their internal organs left-right inverted, with the heart on the right. The findings originally seemed completely mysterious; but similar effects are seen in mammals with other mutations resulting in defective cilia. This suggests that ciliary beating somehow controls which way the left-right axis is oriented.
Time-lapse videomicroscopy in the living mouse embryo reveals that the cells at the node, on its internal face, have cilia that beat in a helical fashion: like a screw-thread, they have a definite handedness, and at the node they are set in a little hollow that is shaped so that their beating drives a current of fluid towards the left side (see Figure 21-82A). Signal proteins carried in this current are thought to provide the bias that orients the left-right axis of the mouse body.
The handedness of the ciliary beating reflects the handedness—the left-right asymmetry—of the organic molecules of which all living things are made. It seems that this, therefore, is the ultimate director of the left-right asymmetry of our anatomy.
Summary
Animal development involves dramatic cell movements. Thus, in gastrulation, cells from the exterior of the early embryo tuck into the interior to form a gut cavity and create the three germ layers—endoderm, mesoderm, and ectoderm—from which higher animals are constructed. In vertebrates, the movements of gastrulation are organized by signals from the Organizer (the dorsal lip of the amphibian blastopore, corresponding to the node in a chick or mouse embryo). These signals specify the dorsoventral axis of the body and govern convergent extension, in which the sheet of cells moving into the interior of the body lengthens along the head-to-tail axis while narrowing at right angles to this axis. The active repacking movements of individual cells that drive convergent extension are coordinated through the Frizzled/Dishevelled planar-polarity signaling pathway—a branch of the Wnt signaling pathway that regulates the actin cytoskeleton.
Subsequent development involves many further cell movements. Part of the ectoderm thickens, rolls up, and pinches off to form the neural tube and neural crest. In the midline, a rod of specialized cells called the notochord elongates to form the central axis of the embryo. The long slabs of mesoderm on either side of the notochord become segmented into somites. Migrant cells, such as those of the neural crest, break loose from their original neighbors and travel through the embryo to colonize new sites. Specific cell-adhesion molecules, such as cadherins and integrins, help to guide the migrations and control the selective cohesion of cells in new arrangements.
Ultimately, the pattern of cell movements is directed by the pattern of gene expression, which determines cell surface properties and motility. Thus, the formation of somites depends on a periodic pattern of gene expression, which is laid down by a biochemical oscillator in the mesoderm and dictates the way the mass of cells will break up into separate blocks. Similarly, the left-right anatomical asymmetry of the vertebrate body is foreshadowed by left-right asymmetry in the pattern of gene expression in the early embryo. This asymmetry, in mammals at least, is thought to be directed ultimately by the handedness of ciliary beating in the neighborhood of the node.
- The Polarity of the Amphibian Embryo Depends on the Polarity of the Egg
- Cleavage Produces Many Cells from One
- Gastrulation Transforms a Hollow Ball of Cells into a Three-Layered Structure with a Primitive Gut
- The Movements of Gastrulation Are Precisely Predictable
- Chemical Signals Trigger the Mechanical Processes
- Active Changes of Cell Packing Provide a Driving Force for Gastrulation
- Changing Patterns of Cell Adhesion Molecules Force Cells Into New Arrangements
- The Notochord Elongates, While the Neural Plate Rolls Up to Form the Neural Tube
- A Gene-Expression Oscillator Controls Segmentation of the Mesoderm Into Somites
- Embryonic Tissues Are Invaded in a Strictly Controlled Fashion by Migratory Cells
- The Distribution of Migrant Cells Depends on Survival Factors as Well as Guidance Cues
- Left-Right Asymmetry of the Vertebrate Body Derives From Molecular Asymmetry in the Early Embryo
- Summary
- Cell Movements and the Shaping of the Vertebrate Body - Molecular Biology of the...Cell Movements and the Shaping of the Vertebrate Body - Molecular Biology of the Cell
- Facts and Figures Concerning the Human Retina - WebvisionFacts and Figures Concerning the Human Retina - Webvision
- argB [Escherichia coli str. K-12 substr. MG1655]argB [Escherichia coli str. K-12 substr. MG1655]Gene ID:948464Gene
- SPATA31A1 SPATA31 subfamily A member 1 [Homo sapiens]SPATA31A1 SPATA31 subfamily A member 1 [Homo sapiens]Gene ID:647060Gene
- ST20 suppressor of tumorigenicity 20 [Homo sapiens]ST20 suppressor of tumorigenicity 20 [Homo sapiens]Gene ID:400410Gene
Your browsing activity is empty.
Activity recording is turned off.
See more...