NCBI Bookshelf. A service of the National Library of Medicine, National Institutes of Health.
Riddle DR, editor. Brain Aging: Models, Methods, and Mechanisms. Boca Raton (FL): CRC Press/Taylor & Francis; 2007.
I. INTRODUCTION
It is now well known that one generally experiences relatively mild changes in cognitive abilities with age, particularly with abilities such as short-term memory, executive functions, and confrontation naming [1]. However, a select group of these “successfully” aged individuals evidence virtually no change in their cognitive abilities with age, even into the eleventh decade of life [2]. Such individuals have often been referred to as examples of “pristine” successful aging. At the other end of the continuum, a large percentage of people are known to develop marked cognitive decline with age, characterized by a dementia state, with a majority of those developing Alzheimer’s disease (AD). These individuals fall into the category of “unsuccessful” aging. In recent years, however, clinical researchers have characterized a group as individuals who, with advancing age, show a moderate impairment in one or more cognitive domains that affects the ability to carry out activities of daily living but does not reach the threshold of a dementia state. This category, classified by many as “mild cognitive impairment” (MCI), reasonably can be regarded as a second general category of “unsuccessful” aging. Although many consider MCI as the earliest stage of Alzheimer’s disease, evidence suggests that MCI represents a separate static and chronic state of normal aging. The etiology of MCI is unknown, but stroke and heart disease risk factors, genetics, and education have all been raised as possible contributors.
While nongenetically altered laboratory animals do not evidence the brain changes seen in AD [3], they have reliably demonstrated age-related changes in cognitive function. Evidence from a wide range of studies in rodents, dogs, and non-human primates have shown as a group that aged subjects are significantly impaired relative to young controls on cognitive tasks that assess functions such as working memory, declarative memory, and executive function: the same functions that evidence impairment in human aging [4–16]. However, on close inspection of the data, the degree of impairment within the aged group is anything but uniform and, in fact, is often dichotomous. While the overall group effect yields an impairment in the given function, it is clear that individuals within the aged group evidence only mild or, in some cases, no impairment on the task while others demonstrate severe impairment.
The finding of individual differences, emphasized in the rodent literature (see [17]), has not been addressed previously in studies of nonhuman primates. This is due in part to the small number of studies in aging primates as well as the relatively small sample sizes of aged groups available within studies. This chapter presents data that we have collected over the past 15 years as part of an ongoing study using the rhesus monkey as a model of normal human aging. During this period, more than 125 rhesus monkeys ranging in age from 5 to 31 years of age have been assessed on multiple tests of recognition memory and executive function. In this chapter we present the data from these animals and discuss their varying levels of impairments and how this relates to clinical findings in human studies.
II. NONHUMAN PRIMATES AS SUITABLE ANIMAL MODELS OF AGING
Several hypotheses have been advanced to account for the neural bases that underlie the cognitive decline that occurs in normal, disease-free aging, but the processes underlying such decline are still not well understood. This is in large part due to our inability to concurrently collect behavioral, physiological, and pathological data within a short interval of time in human studies. Additional limitations are encountered by problems related to inadequate preservation of tissue, as well as the usual problems of control over extraneous variables. Given these limitations, it is essential to use a suitable animal model of normal human aging to address some of these questions. In a recent symposium on aging and Alzheimer’s disease, a number of investigators reiterated the value of non-human primates as an animal model of human aging (Assessing Cognition for Emerging Therapeutics in AD, Johns Hopkins School of Medicine, September 2004). Rhesus monkeys have a rich and well-studied behavioral repertoire that is well suited for these types of investigations of normal aging, as they do not develop Alzheimer’s disease. In addition, with this animal model, not only is it possible to collect a wide range of behavioral, morphological, biochemical, and molecular data within a short period of time, but opportunities are also provided for the exploration of treatment modes and interventions to arrest or reverse age-related cognitive decline.
III. COGNITIVE ASSESSMENT OF THE AGED NONHUMAN PRIMATE
As discussed above, monkeys have a rich behavioral repertoire that lends itself suitably to translational studies of human cognition. Indeed, several behavioral tasks developed in the human neuropsychological domain have now been successfully adapted to the nonhuman primate. In some instances, tasks developed for non-human primate studies have been incorporated into both research and clinical test batteries for human studies. This has occurred primarily in the domains of memory, executive function, and attention. The following subsections outline each of these domains as they relate to nonhuman primates.
A. Memory
Perhaps the best-characterized change that occurs in normal aging is a decline in short-term memory function. The literature on memory dysfunction in human aging is voluminous and several reviews and books have been devoted to the subject (e.g., [18–21]). They show that changes in memory begin as early at the fifth decade of life, that is, middle age. This is supported by recent data from our long-standing study of normal aging in the rhesus monkey that indicates that memory changes also occur in early middle age in the monkey. Another characteristic of memory function that is impaired in normal human aging is the recall of information. The impairment in recall is greater than that observed in recognition memory, and this relationship appears to be independent of the type of stimulus material used [22–25]. Rabinowitz [26] reported a threefold impairment in recall relative to recognition even under conditions of cueing. Of interest, the rate of forgetting, often accelerated in many neurodegenerative disorders, does not appear to change with age [27, 28]. However, there is some evidence that older individuals show some degradation in recall when memory is assessed over very long delay intervals [29]. Deficits on tasks with increasing delays imposed between stimulus presentation and response trials have also been observed in aged monkeys. Using a delayed response procedure, several investigators [14, 30–33] have shown reduced memory by monkeys 18 years of age or older relative to young adult monkeys. Further, the degree of impairment appears related to the length of delay.
Since the seminal studies by Bartus and colleagues [14], evidence has accumulated from several laboratories demonstrating impairment in recognition memory in aged monkeys [4, 10, 11, 33–39]. A related series of studies has suggested that spatial memory appears adversely affected by the aging process, whereas object recognition is only mildly affected [4, 10, 11, 33–40]. In another study, we extended this finding by administering a spatial and a non-spatial form of the delayed recognition span test, a test of memory span, to groups of young adult and early senescent rhesus monkeys. The results of this study showed that aged animals were more impaired on the spatial than on the nonspatial stimulus conditions [35].
B. Executive Function
While there exists an impressive literature on age-related decline in memory with normal aging and age-related disease, the number of studies aimed at the assessment of executive function is more limited. Executive function is the term applied to such abilities as set-shifting, abstraction, and response suppression. Set-shifting refers to the capacity to keep track or correctly identify correct response-reward associations in the face of frequently changing contingencies. Abstraction refers to the capacity to identify a common element among stimuli that differ along several dimensions. Response inhibition refers to the ability to suppress previous response patterns to permit testing of new ones. Deficits in executive function have been attributed for the most part to dorsolateral prefrontal dysfunction [41–43].
In nonhuman primates, studies of frontal lobe function, the area of the cortex involved in mediating executive function and working memory, date back to the 1930s with Jacobsen’s classical studies of the delayed response task in the chimpanzee [44]. Over the past 50 years, several investigators have confirmed and extended these early findings in a variety of species, including the monkey [45–49], and have demonstrated impairments on a variety of tasks with damage in various areas of the frontal lobe [47, 50–52].
More recently, in an attempt to closely approximate clinical studies of normal human aging with our nonhuman primate model, we have adapted a well-established human test of executive function, the Wisconsin Card Sorting Test (WCST) [53, 54] for use with nonhuman primates. Similar efforts to develop tests for monkeys that use the conceptual framework of those used with humans have been advanced by other laboratories. Dias and colleagues [55] assessed attentional set-shifting in primates with frontal lobe lesions as part of their CANTAB program software for testing both humans and monkeys. Our test, the Conceptual Set Shifting Task (CSST), more closely resembles the human WCST by using the color and shape stimuli and learning criteria of the WCST and requires the monkey to establish a pattern of responding to a concept based on a reward contingency, maintain responding to that concept for a period of time, and then shift to a different concept as the reward contingency changes [5, 56]. It thus assesses both abstraction and set-shifting in a way exactly analogous to the WCST. Moreover, the CSST has been shown to be sensitive to damage to the dorsolateral prefrontal cortex [43].
In normal aged humans, a variety of studies have reported impairments on tasks of executive function [1, 57–63], including specific age-related deficits in abstraction [64, 65] and set-shifting [63]. Caution in interpreting some of these results has been raised by some investigators [66] who suggest that deficits in abstraction may be exacerbated by tasks that make heavy demands on memory and attention. This concern was addressed in a later study that revealed impairments on tasks of abstraction and set shifting that had low memory and attentional demands in optimally healthy aged subjects [63].
IV. STUDIES ON SPECIFIC TASKS OF MEMORY AND EXECUTIVE FUNCTION
The behavioral tasks used in our studies were derived from two sources. The first source was a battery of learning, memory, and cognitive flexibility tasks that have been used as “benchmark” tests of function in monkeys with selective limbic system and frontal cortical lesions. Some of these tasks were subsequently adapted for use with normal aged humans and to patients with Alzheimer’s disease, frontal lobe dementia, and a variety of amnestic disorders [67, 68]. The second source of tests came from those used in standard human neuropsychological testing that have been modified for use with monkeys such as the CSST [69]. Together this battery of tasks offers the advantage that (1) it can be administered and performed by aged human and nonhuman primates alike; (2) the tasks are carefully controlled and allow analyses of performance patterns that can differentiate among subjects, both human and monkey, with different neuropathologies; and (3) there exists an accumulated wealth of information on structure-function relationships from human and animal studies assessing and carefully characterizing the effects of specific brain damage using these tasks.
In our cohort, animals range in age from 5 to 31 years of age. Based on an extensive survival study at Yerkes Regional Primate Research Center, which suggests a 1:3 ratio between monkey and human years of age, we have characterized young monkeys as 5 to 10 years of age, middle-aged monkeys as 13 to 19 years of age, early-aged monkeys as 20 to 24 years of age, and advanced-aged monkeys as >25 years of age.
A. Definition of Cognitive Impairment
Within this group of animals, we developed a cognitive impairment index (CII) as a measure of cognitive function in aging. This measure provides a convenient index for ranking and comparing individual animals. The CII was derived based on the principal components analysis [4], which indicated that overall impairments were predicted by a weighted average of each subject’s scores on the DNMS, the DNMS-2 minute delay, and DRST-spatial (see test descriptions below). To simplify this, the scores on each of the three tasks for each individual were converted to a z-score relative to the mean performance of young adults (the baseline reference group). The CII was then computed as a simple average of the three standardized scores, with positive numbers indicating increasing impairments in z-units from the mean of the baseline reference group of young adult monkeys.
With this index, we established a cutoff for mild impairment as a CII that is 1.0 to 2.0 standard deviations above the mean for young adult monkeys. Thus, monkeys with a CII 1.0 to 2.0 SD above the mean of the young animals are considered to be mildly impaired, whereas monkeys with CIIs of 2.0 SDs or more above the mean of the young are considered severely impaired. Monkeys with CIIs below 1.0 are considered in the nonimpaired range. Based on these criteria for levels of impairment, we determine that animals in the normal range of performance and in the mild impairment range are equivalent to what has been termed “successful aging” in the human literature. Similarly, animals that fall into the severely impaired range are equivalent to “unsuccessful aging,” as observed in human clinical studies.
Within our cohort of animals, based on the CII measurement, 4.55% of animals in the 13- to 19-year-old age group were mildly impaired and 22.7% were severely impaired. In the 20- to 24-year-old age range, 20.69% of the animals were mildly impaired and 20.69% of the animals were severely impaired. Finally, 19.35% of the animals in the 25- to 31-year-old age range were mildly impaired while 32.3% were severely impaired (Table 2.1). Overall, the percent of animals in the mild impairment range remained relatively stable through middle age and advanced age but the percent of animals in the severely impaired range (unsuccessful aging) increased by almost 15% from middle age and advanced age. As expected, the overall number of animals demonstrating significant cognitive impairment increased with age but there were still a considerable number of animals with mild or no cognitive impairment (Figure 2.1).
TABLE 2.1
Cognitive Impairment Index
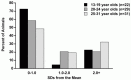
FIGURE 2.1
Cognitive impairment index: percent of animals in the normal, mild impairment, and unsuccessful ranges.
While the CII is an important measure of cognitive function, we thought it was important to also look at animals’ performance on individual tests of memory and executive function. Using the same criterion as with the CII for level of impairment (mild impairment = 1.0–2.0 SD from the mean of the young on each test; and severe impairment equal to or greater than 2.0 SD from the mean of the young on each test), we determined the percent of animals that fall within the normal, mildly impaired, and severely impaired range on each individual test in our study. The results are outlined in the subsections below.
B. Delayed Nonmatching to Sample (DNMS)
1. Overview of DNMS
The DNMS task is a benchmark recognition memory task that assesses the subject’s ability to identify a novel stimulus from a familiar stimulus following a specific delay interval. Although originally developed for assessment of memory in animals, it also has been used with human subjects. Various forms of this task have been used to assess memory function in monkeys following specific lesions of the forebrain, including transection of the fornix [70–72], limited or combined lesions of the hippocampus and amygdala [71–75], or medial dorsal thalamus [76].
This task is administered in three phases. First, in the acquisition phase, the animal must learn the rule of the task to reach criterion. After criterion is reached, a delay component is added where the animal must remember the stimulus over an increasing delay. Finally, in surgical animal models, there is the postoperative relearning phase of the DNMS where the animal must reestablish a level of learning criterion after a surgical procedure. Depending on the experimental paradigm, deficits on the acquisition or post-operative relearning phase of the DNMS task have been reported following damage to the hippocampus [71, 74, 77, 78], and to a greater extent in monkeys with combined damage to the amygdala and hippocampus [73, 74]. More recently, impairments have been found following damage to extrahippocampal regions, including rhinal cortex, entorhinal cortex, perirhinal cortex, and adjacent parahippocampal cortices [79–84], regions that are critical components of temporal lobe neuronal circuitry linking the hippocampus and amygdala with the rest of the forebrain. In humans, the DNMS task also has been shown to be sensitive to selected temporal lobe damage [85] and to a variety amnestic disorders [86], as well as to Alzheimer’s disease and normal aging [67].
In aged monkeys, results from studies in several laboratories have shown an impairment in the acquisition and delay phases of the DNMS task [4, 10, 33, 36, 37, 39, 40]. The findings appear to be quite consistent across these studies and show that, as a group, aged monkeys are mildly impaired on the acquisition and delay conditions of the DNMS task. Another consistent finding is variability in the performance of aged animals. Whereas some aged monkeys evidence impairment, others perform the task as proficiently as young adults [36, 37, 39].
2. Successful vs. Unsuccessful Aging in Performance on DNMS
This pattern of variability in performance on the DNMS task by aged animals is confirmed in the data from our cohort (see Table 2.2). On the acquisition phase, none of the animals in the 13- to 19-year-old age group fell within the mild impairment range (1.0–2.0 SD from the mean) while 21.7% of the animals in this group were in the severe impairment range (greater than 2.0 SD from the mean of the young). Whereas in the 20- to 24-year-old age range, 13.8% and 27.6% of the animals were 1.0–2.0 SD and greater than 2.0 SD, respectively, from the mean of the young. Finally, in the 25- to 31-year-old age range, 9.68% of the animals were mildly impaired and 35.48% were severely impaired. A similar pattern of impairments was observed in the delay components of the DNMS (Table 2.2). It has been suggested that the degree of impairment on the DNMS may be related to the extent of limbic system dysfunction [37, 39]. Consistent with this notion is the suggestion that the nature of the deficit on DNMS in impaired aged monkeys is due to a disproportionate sensitivity to proactive interference [14, 15]. It is of interest that, when aged monkeys were tested on a version of the DNMS task that contains an extensive degree of interference (i.e., using the same pair of objects throughout the task), their performance declined precipitously to a level comparable to that of their performance on the delayed response task [87]. Whether this simply reflects an increase in task difficulty or a shift in the underlying locus of neuronal circuitry is unclear.
TABLE 2.2
Delayed Nonmatching to Sample
C. Delayed Recognition Span Task (DRST)
1. Overview of DRST
This task is a short-term memory test that was designed to investigate recognition memory in monkeys following bilateral removal of the hippocampus [88]. It requires the subject to identify, trial-by-trial, a new stimulus among an increasing array of serially presented, familiar stimuli. The task is administered using different classes of stimulus material, spatial vs. nonspatial (color, pattern, objects), to characterize possible material-specific recognition memory impairments. An expanded form of this task has been used in the same manner to assess recognition in a variety of neurological patient populations including those of Alzheimer’s disease [67, 89], Huntington’s disease or Korsakoff’s disease [68], and Parkinson’s disease [90]. In normal aged human subjects, recognition span memory is impaired in several stimulus classes, including spatial position and visual patterns [68, 91].
2. Successful vs. Unsuccessful Aging in Performance on DRST
In our study, this task is administered using two different classes of stimulus material: spatial location or object shape. This allows us to characterize any recognition memory deficits that may occur as a general impairment or one that is material specific.
a. Delayed Recognition Span Test: Spatial
Unlike the pattern of number of animals considered impaired with increasing age on learning the DNMS basic task, few animals were impaired (1.0–2.0 or greater than 2 Standard Deviations from the mean of the young monkeys) on DRST task. In fact, none of the animals in the 13- to 19-year-old range were impaired, and only 6.89% of animals in the 20- to 24-year-old age range fell within the mild impairment range. None of the animals in this age range were classified as severely impaired. Similarly, 6.45 % and 3.22% of animals in the 25- to 31-year-old range were mildly and severely impaired, respectively, on the spatial DRST (see Table 2.3).
TABLE 2.3
Delayed Recognition Span Test
b. Delayed Recognition Span Test: Object
An almost identical pattern was observed on the object DRST. The performance of a very small number of animals was in the mild and severe impairment ranges (see Table 2.3).
D. Conceptual Set Shifting Task
1. Overview of Conceptual Set-Shifting
Executive function is one of the first cognitive abilities to be compromised in aging and age-related disease [5, 6, 67] and is considered a primary function of the prefrontal cortices [47, 92–94]. As summarized above, we developed a task suitable for testing monkeys based on the principles of the standard frontal lobe set-shifting task used in humans, the Wisconsin Card Sorting Test (WCST) [53, 54]. Our test, the Conceptual Set Shifting Task (CSST), uses a subset of the same stimuli as the WCST to assess abstraction, establishment, maintenance, and shifting of sets and perseveration in a manner that parallels that used in human clinical studies.
2. Successful vs. Unsuccessful Aging in Performance on CSST
Of the 128 animals in our group, 66 were tested on the CSST. Performance on the CSST appears to be affected early in aging and may become the most compromised with advanced age. In contrast to tests of memory where the performance of a few subjects reached the severe impairment range, a large number of animals were in the mild and severe impairment range on the CSST. In fact, on various measures of performance on the CSST, 12 middle-aged animals were mildly impaired and between 25 and 41% were severely impaired. In contrast, in the aged animals, 0 to 32% of the animals were mildly impaired and 0 to 36% were severely impaired (Table 2.4). These findings would suggest that executive system functions may be among the most sensitive domains affected with advancing age.
TABLE 2.4
Conceptual Set-Shifting Task
E. Summary of Behavioral Findings
Based on the performance of these 128 monkeys on two tests of recognition memory, one test of executive function and a composite score of cognitive function, we have demonstrated a pattern of variable levels of performance on these tasks. While overall as groups, the 20- to 24- and 25- to 31-year-olds are impaired on these tests, there is considerable variability in performance. Many animals show no impairment and few show mild to severe impairments on tests of memory. On a test of executive function, more animals demonstrate mild and severe impairments, but still more than 50% show no impairment. This pattern of variability in performance is similar to what is observed in human clinical studies. In human studies, those individuals demonstrating no impairment (equivalent to scores below 1.0 SD in our study) might be considered among the more pristine among those successfully aging. While those demonstrating mild impairments (1.0 to 2.0 SD above the mean of the young in our study) might be considered to be more typically those who are successfully aging, but evidence some degree of cognitive decline associated with typical aging, and finally those demonstrating significant cognitive impairment (greater than 2.0 SD from the mean of the young in our study) are deemed as “unsuccessful aging” and may reflect the human classification of “mild cognitive impairment,” keeping in mind that monkeys do not develop the pathological or behavioral profile of Alzheimer’s disease [95].
V. NEUROBIOLOGICAL BASIS FOR COGNITIVE DECLINE
Several possibilities have been advanced to account for mild or marked age-related cognitive decline, ranging from widespread cortical neuronal loss and neurotransmitter depletion, to amyloid deposition and to the development of neuritic plaques. While likely contributing to cognitive dysfunction, we believe that these factors are unlikely primary candidates to account for age-related cognitive decline. With few exceptions, neurons in the cerebral cortex do not undergo marked loss [95] and the presence and the extent of neuritic plaques or amyloid burden are quite variable and are not correlated with cognitive decline [96]. Rather, we have accumulated evidence over the past several years that lead us to the view that alteration and loss of white matter may be the principal neurobiological change that underlies age-related cognitive decline [3]. In electron microscopic (EM) studies of the effects of aging in the cerebral cortex [97, 98], corpus callosum [98], and optic nerve [99] of monkeys, myelin sheaths have been found to show marked age-related changes, including the accumulation of dense cytoplasm and the formation of fluid-filled balloons. In addition, the formation of sheaths with redundant myelin and thick sheaths occur with continued formation of myelin with age [100]. Of particular interest, the frequency of these alterations in myelin with age correlates significantly with the cognitive decline exhibited by monkeys. In support of this, using diffusion tensor magnetic resonance imaging (DT-MRI), we have recently reported significant age-related loss of fractional anisotropy (FA) in forebrain white matter of the frontal lobe. Reduced FA is regarded as a marker of white matter abnormalities and, like the EM measures, correlates with cognitive decline [101]. Further, unpublished data from our group on conduction across the corpus callosum indicates that, with age, there is a significant alteration in the profile of conduction parameters. This result could be explained if the myelin dystrophy observed in the corpus callosum [98] disrupts conduction in a fraction of the nerve fibers and suggests that alterations in myelin integrity and consequent disruption of conduction may alter the signal strength and hence the information transfer that is critical for neuronal circuits to operate properly. Finally, using designed-based stereology and MRI in separate studies, our group has demonstrated nerve fiber loss with age from the optic nerves [99], a 40% loss of nerve fibers in the anterior commissure of aged rhesus monkeys, and a significant loss of white matter volume on with MRI, with an accompanying increase in ventricular size (Wisco, Killiany, and Rosene, unpublished observations). These observations lend further support to the notion that there is likely to be a global loss of myelinated nerve fibers with age. Extensive multidisciplinary investigations into the precise mechanisms of these age-related changes in white matter and their relationship to cognitive decline are the focus of our current studies with the continued use of our nonhuman primate model of normal aging.
ACKNOWLEDGMENTS
The authors wish to acknowledge support from NIH grants PO1-AG0001 (DLR), R37-AG17609 (MBM), and R55-AG12610.
REFERENCES
- 1.
- Albert MS. Neuropsychological and neurophysiological changes in healthy adult humans across the age range. Neurobiol. Aging. 1993;14:623. [PubMed: 8295666]
- 2.
- Perls TT. The different paths to 100. Am. J. Clin. Nutr. 2006;83:484S. [PubMed: 16470017]
- 3.
- Peters A, Rosene DL. In aging, is it gray or white. J. Comp. Neurol. 2003;462:139. [PubMed: 12794738]
- 4.
- Herndon JG, et al. Patterns of cognitive decline in aged rhesus monkeys. Behav. Brain Res. 1997;87:25. [PubMed: 9331471]
- 5.
- Moore TL, et al. Impairment in abstraction and set shifting in aged rhesus monkeys. Neurobiol. Aging. 2003;24:125. [PubMed: 12493558]
- 6.
- Moore TL, et al. Neurobiol Aging. 2006. Executive system dysfunction occurs as early as middle-age in the rhesus monkey. in press. [PubMed: 16183172]
- 7.
- Lasarge CL, et al. Deficits across multiple cognitive domains in a subset of aged Fischer 344 rats. Neurobiol Aging. 2006 June 22 [PubMed: 16806587]
- 8.
- Tapp PD, et al. Frontal lobe volume, function, and beta-amyloid pathology in a canine model of aging. J. Neurosci. 2004;24:8205. [PMC free article: PMC6729694] [PubMed: 15385603]
- 9.
- Tapp PD, et al. Concept abstraction in the aging dog: development of a protocol using successive discrimination and size concept tasks. Behav. Brain Res. 2004;153:199. [PubMed: 15219721]
- 10.
- Bachevalier J. Behavioral changes in aged rhesus monkeys. Neurobiol. Aging. 1993;14:619. [PubMed: 8295665]
- 11.
- Moss MB, et al. Recognition span in aged monkeys. Neurobiol. Aging. 1997;18:13. [PubMed: 8983028]
- 12.
- Arnsten AFT, Jentsch JD. The alpha-1 adrenergic agonist, cirazoline, impairs spatial working memory performance in aged monkeys. Pharmacol. Biochem. Behavior. 1997;58:55. [PubMed: 9264070]
- 13.
- Arnsten AFT, et al. Dopamine D1 receptor mechanisms in the cognitive performance of young adult and aged monkeys. Psychopharmacology. 1994;116:143. [PubMed: 7862943]
- 14.
- Bartus JM, Fleming D, Johnson HR. Aging in the rhesus monkey: debilitating effects on short-term memory. J. Gerontol. 1978;34:209. [PubMed: 106081]
- 15.
- Bartus RT, Dean RL. Recent memory in aged non-human primates: hypersensitivity to visual interference during retention. Expl. Aging Res. 1979;5:385. [PubMed: 118012]
- 16.
- Bartus RT, Dean RL, Fleming DL. Aging in the rhesus monkey: effects on visual discrimination learning and reversal learning. J. Gerontol. 1979;34:209. [PubMed: 108323]
- 17.
- Baxter MG, Gallagher M. Neurobiological substrates of behavioral decline: models and data analytic strategies for individual differences in aging. Neurobiol. Aging. 1996;17:491. [PubMed: 8725914]
- 18.
- Poon LW. Differences in human memory with aging: nature, causes and clinical implications. In: Birren JE, Shaie KW, editors. Handbook of the Psychology of Aging. Van Nostrand Reinhold; New York: 1985. p. 427.
- 19.
- Light L. Memory and aging: four hypotheses in search of data. Annu. Rev. Psychol. 1991;42:333. [PubMed: 2018397]
- 20.
- Albert MS. Age-related changes in cognitive function. In: Albert MA, Knoefel JE, editors. Clinical Neurology of Aging. Oxford University Press; New York: 1994. p. 314.
- 21.
- Powell DH. Profiles in Cognitive Aging. Harvard University Press; Cambridge, MA: 1994.
- 22.
- Schonfield D, Robertson BA. Memory storage and aging. Canad. J. Psych. 1966;20:228. [PubMed: 5942327]
- 23.
- Harwood E, Naylor GFK. Recall and recognition in elderly and young subjects. Austr. J. Psych. 1969;21:251.
- 24.
- Howell SC. Familiarity and complexity in perceptual recognition. J. Gerontol. 1972;27:364. [PubMed: 5046606]
- 25.
- Erber JT. Age differences in recognition memory. J. Gerontol. 1974;29:177. [PubMed: 4811953]
- 26.
- Rabinowitz J. Priming in episodic memory. J. Gerontol. 1986;41:204. [PubMed: 3950347]
- 27.
- Talland GA. Age and the immediate memory span. Gerontologist. 1967;7:4. [PubMed: 6041903]
- 28.
- Schonfield D. Age and remembering. Proc Sem, Duke University Council on Aging and Human Development; Durham, NC: 1969.
- 29.
- Park D, et al. Forgetting of pictures over a long retention interval in old and young adults. Psychol. Aging. 1988;3:94. [PubMed: 3268247]
- 30.
- Kubo N, et al. Behavioral compensations in a positional learning and memory task by aged monkeys. Behav. Processes. 2001;56:15. [PubMed: 11566233]
- 31.
- Marriott JG, Abelson JS. Age differences in short-term memory of test-sophisticated rhesus monkeys. Age. 1980;3:7.
- 32.
- Medin DL, Davis RT. Shrier AM, Stollmitz F, editors. Academic Press; New York: Behavior of Non-Human Primates. 1974:1.
- 33.
- Rapp PR, Amaral DG. Evidence for a task-dependent memory dysfunction in the aged monkey. J. Neurosci. 1989;9:3568. [PMC free article: PMC6569903] [PubMed: 2795141]
- 34.
- Calhoun ME, et al. Reduction in hippocampal cholinergic innervation is unrelated to recognition memory impairment in aged rhesus monkeys. J. Comp. Neurol. 2004;475:238. [PubMed: 15211464]
- 35.
- Killiany RJ, et al. Recognition memory function in early senescent rhesus monkeys. Psychobiology. 2000;28:45.
- 36.
- Presty SK, et al. Age differences in recognition memory of the rhesus monkey (Macaca mulatta). Neurobiol. Aging. 1987;8:435. [PubMed: 3683724]
- 37.
- Moss MB, Rosene DL, Peters A. Effects of aging on visual recognition memory in the rhesus monkey. Neurobiol. Aging. 1988;9:495. [PubMed: 3062461]
- 38.
- Bachevalier JL, et al. Aged monkeys exhibit behavioral deficits indicative of widespread cerebral dysfunction. Neurobiol. Aging. 1991;12:99. [PubMed: 2052134]
- 39.
- Rapp PR, Amaral DG. Recognition memory deficits in a subpopulation of aged monkeys resemble the effects of medial temporal lobe damage. Neurobiol. Aging. 1991;12:481. [PubMed: 1770984]
- 40.
- Arnsten AFT, Goldman-Rakic PS. Analysis of α-2 adrenergic agonist effects on the delayed nonmatch-to-sample performance of aged rhesus monkeys. Neurobiol. Aging. 1990;11:583. [PubMed: 1980719]
- 41.
- Stuss DT. Interference effects on memory function in posteukotomy patients: an attentional perspective. In: Levin HS, Eisenberg HM, Benton AL, editors. Frontal Lobe Function and Dysfunction. Oxford University Press; Oxford: 1991. p. 157.
- 42.
- Shimamura AP, Janowsky JS, Squire LR. What is the role of frontal lobe damage in memory disorders? In: Levin HS, Eisenberg HM, Benton AL, editors. Frontal Lobe Function and Dysfunction. Oxford University Press; Oxford: 1991. p. 173.
- 43.
- Moore TL, et al. Lesions of dorsal prefrontal cortex produce an executive function deficit in the rhesus monkey. Soc Neurosci Abst. 2001;27 Prog. #533.6.
- 44.
- Jacobsen CF. An experimental analysis of the frontal association areas in primates. Arch. Neurol. Psychiat. 1935;33:558.
- 45.
- Mishkin M. Effects of small frontal lesions on delayed alternation in monkeys. J. Neurophysiol. 1957;20:615. [PubMed: 13476217]
- 46.
- Butter CM, Mishkin M, Mirsky AF. Emotional responses toward humans in monkeys with selective frontal lesions. Physiol. Behav. 1968;3:213.
- 47.
- Goldman PS, Rosvold HE. Localization of function within the dorsolateral prefrontal cortex of the rhesus monkey. Exp. Neurol. 1970;27:291. [PubMed: 4987453]
- 48.
- Gross CG. Comparison of the effects of partial and total lateral frontal lesions on test performance by monkeys. J. Comp. Physiol. Psychol. 1963;56:41. [PubMed: 13950748]
- 49.
- Iversen SD. Interference and inferotemporal memory deficits. Brain Res. 1970;19:277. [PubMed: 4988263]
- 50.
- Battig K, Rosvold HE, Mishkin M. Comparison of the effects of frontal and caudate lesions on delayed response and alternation in monkeys. J. Comp. Physiol. Psych. 1960;53:400. [PubMed: 13797573]
- 51.
- Goldman PS, et al. Analysis of the delayed-alternation deficit produced by dorsolateral prefrontal lesions in the rhesus monkey. J. Comp. Physiol. Psychol. 1971;77:212. [PubMed: 5000659]
- 52.
- Pohl WG. Dissociation of spatial discrimination deficits following frontal and parietal lesions in monkey. J. Comp. Physiol. Psychol. 1973;82:227. [PubMed: 4632974]
- 53.
- Berg EA. A simple objective test for measuring flexibility in thinking. J. General Psychol. 1948;39:15. [PubMed: 18889466]
- 54.
- Grant DA, Berg EA. A behavioral analysis of degree of reinforcement and ease of shifting to new responses in a Weigl-type card sorting problem. J. Exp. Psychol. 1948;34:404. [PubMed: 18874598]
- 55.
- Dias R, Robbins TW, Roberts AC. Primate analogue of the Wisconsin Card Sorting Test: effects of excitotoxic lesions of the prefrontal cortex in the marmoset. Behav. Neurosci. 1996;110:872. [PubMed: 8918991]
- 56.
- Moore TL, et al. Impairment of executive function induced by hypertension in the rhesus monkey (Macaca mulatta). Behav. Neurosci. 2002;116:387. [PubMed: 12049319]
- 57.
- Royall DR. Executive cognitive impairment: a novel perspective on dementia. Neuroepidemiology. 2000;19:293. [PubMed: 11060503]
- 58.
- Tierney MC. Cognitive tests that best discriminate between presymptomatic AD and those who remain nondemented. Neurology. 2001;57:163. [PubMed: 11445658]
- 59.
- Daigneault S, Braun CM, Whitaker HA. Early effects of normal aging on perseverative and non-perseverative prefrontal measures. Develop. Neuropsych. 1992;8:99.
- 60.
- Talland GA. Effects of aging on the formation of sequential and spatial concepts. Percept. Mot. Skills. 1961;13:210.
- 61.
- Albert MS, Duffy FH, Naeser MA. Nonlinear changes in cognition and their neurophysiological correlates. Canad J Psych. 1987;41:141. [PubMed: 3502893]
- 62.
- Lezak M. Neuropsychological Assessment. 2. Oxford University Press; New York: 1983.
- 63.
- Albert MS, Wolfe J, Lafleche G. Differences in abstraction ability with age. Psych. and Aging. 1990;5:94. [PubMed: 2317307]
- 64.
- Bromley D. Effects of age on intellectual output. J. Gerontol. 1957;12:318. [PubMed: 13463303]
- 65.
- Mack JL, Carlson NJ. Conceptual deficits and aging: the category test. Percept. Motor Skills. 1978;46:123. [PubMed: 643469]
- 66.
- Hess TM, Slaughter SL. Aging effects on prototype abstraction and concept identification. J. Gerontol. 1986;41:214. [PubMed: 3950348]
- 67.
- Albert MS, Moss MB. The assessment of memory disorders in patients with Alzheimer’s disease. In: Squire L, Butters N, editors. Neuropsychology of Memory. Guilford Press; New York: 1984.
- 68.
- Moss MB, Rosene DL, Peters A. The effects of aging on visual recognition memory in the rhesus monkey. Primate Report. 1986;14:133. [PubMed: 3062461]
- 69.
- Moore TL, et al. A non-human primate test of abstraction and set shifting: an automated adaptation of the Wisconsin card sorting test. J Neurosci Meth. 2005;146:165. [PubMed: 16054506]
- 70.
- Gaffan D. Recognition impaired and association intact in the memory of monkeys after transection of the fornix. J. Comp. Physiol. Psychol. 1974;86:1100. [PubMed: 4209603]
- 71.
- Mahut H, Zola-Morgan S, Moss MB. Hippocampal resections impair associative learning and recognition memory in the monkey. J. Neurosci. 1982;2:1214. [PMC free article: PMC6564312] [PubMed: 7119874]
- 72.
- Saunders RC, Murray EA, Mishkin M. Further evidence that amygdala and hippocampus contribute equally to recognition memory. Neuropsychologia. 1984;22:758. [PubMed: 6527768]
- 73.
- Mishkin M. Memory in monkeys severely impaired by combined but not by separate removal of amygdala and hippocampus. Nature. 1978;273:297. [PubMed: 418358]
- 74.
- Zola-Morgan S, Squire LR. Medial temporal lesions in monkeys impair memory on a variety of tasks sensitive to human amnesia. Behav. Neurosci. 1985;99:22. [PubMed: 4041230]
- 75.
- Alverez P, Zola-Morgan S, Squire LR. Damage limited to the hippocampal region produces long-lasting memory impairment in monkeys. J. Neurosci. 1995;15:3796. [PMC free article: PMC6578197] [PubMed: 7751947]
- 76.
- Aggleton JP, Mishkin M. Visual recognition impairment following medial thalamic lesions in monkeys. Neuropsychologia. 1983;21:189. [PubMed: 6877575]
- 77.
- Zola-Morgan S, Squire LR. Memory impairment in monkeys following lesions limited to the hippocampus. Behav. Neurosci. 1986;100:155. [PubMed: 3964416]
- 78.
- Beason-Held LL, et al. Hippocampal formation lesions produce memory impairment in the rhesus monkey. Hippocampus. 1999;9:562. [PubMed: 10560927]
- 79.
- Murray EA, Mishkin M. Visual recognition in monkeys following rhinal cortical ablations combined with either amygdalectomy or hippocampectomy. J Neurosci. 1986;6:1991. [PMC free article: PMC6568582] [PubMed: 3734871]
- 80.
- Gaffan D, Murray EA. Monkeys (Macaca fascicularis) with rhinal cortex ablations succeed in object discrimination learning despite 24-hr intertrial intervals and fail at matching to sample despite double sample presentations. Behav. Neurosci. 1992;106:30. [PubMed: 1554436]
- 81.
- Meunier M, et al. Effects on visual recognition of combined and separate ablations of the entorhinal and perirhinal cortex in rhesus monkeys. J. Neurosci. 1993;13:5418. [PMC free article: PMC6576426] [PubMed: 8254384]
- 82.
- Eacott MJ, Gaffan D, Murray EA. Preserved recognition memory for small sets, and impaired stimulus identification for large sets, following rhinal cortex ablations in monkeys. Eur J Neurosci. 1994;6:1466. [PubMed: 8000570]
- 83.
- Zola-Morgan S, Squire LR, Amaral DG. Lesions of the hippocampal formation but not lesions of the fornix or the mammillary nuclei produce long-lasting memory impairments in monkeys. J. Neurosci. 1989;9:898. [PMC free article: PMC6569954] [PubMed: 2494309]
- 84.
- Zola-Morgan S, et al. Lesions of perirhinal and parahippocampal cortex that spare the amygdala and hippocampal formation produce severe memory impairment. J Neurosci. 1989;9:4355. [PMC free article: PMC6569635] [PubMed: 2593004]
- 85.
- Comparet P, Darriet D, Jaffard R. Demonstration of dissociation between frontal and temporal lesions in man on two versions of delayed non-matching recognition tests used in monkeys. CR Acad. Sci. 1992;314:515. [PubMed: 1521171]
- 86.
- Squire LR, Zola-Morgan S, Chen KS. Human amnesia and animal models of amnesia: performance of amnesic patients on tests designed for the monkey. Behav. Neurosci. 1988;102:210. [PubMed: 3130073]
- 87.
- Rapp PR. Neuropsychological analysis of learning and memory in the aged non-human primate. Neurobiol. Aging. 1993;14:627. [PubMed: 8295667]
- 88.
- Rehbein L. Long-Term Effects of early Hippocampectomy in the Monkey, unpublished doctoral thesis. Northwestern University; Boston: 1985.
- 89.
- Salmon DP, et al. Recognition memory span in mild and moderately demented patients with Alzheimer’s disease. J. Clin. Exp. Neuropsych. 1989;4:429. [PubMed: 2760179]
- 90.
- Lange KW, et al. L-dopa withdrawal in Parkinson’s disease selectively impairs cognitive performance in tests sensitive to frontal lobe function. Psychopharmacology. 1992;107:394. [PubMed: 1615139]
- 91.
- Inouye SK, et al. Cognitive performance in a high-functioning community-dwelling elderly population. J. Gerontol. 1993;48:146. [PubMed: 8315227]
- 92.
- Milner B. Disorders of memory after brain lesions in man. Preface: material-specific and generalized memory disorder. Neuropsychologia. 1968;6:175.
- 93.
- Milner B. Aspects of human frontal lobe. In: Jasper HH, Riggio S, Goldman-Rakic PS, editors. Function Epilepsy and the Functional Anatomy of the Frontal Lobe. Raven Press; New York: 1995.
- 94.
- Mishkin M. Perseveration of central sets after frontal lesions in monkeys. In: Warren JM, Akert K, editors. The Frontal Granular Cortex and Behavior. McGraw-Hill; New York: 1964. pp. 219–241.
- 95.
- Peters A, et al. Are neurons lost from the primate cerebral cortex during normal aging? Cereb. Cortex. 1998;8:295. [PubMed: 9651126]
- 96.
- Sloan JA, et al. Lack of correlation between plaque burden and cognition in the aged monkey. Acta Neuropath. 1997;94:471. [PubMed: 9386780]
- 97.
- Peters A, Moss MB, Sethares C. Effects of aging on myelinated nerve fibers in monkey primary visual cortex. J. Comp. Neurol. 2000;419:364. [PubMed: 10723011]
- 98.
- Peters A, Sethares C. Aging and the myelinated fibers in prefrontal cortex and corpus callosum of the monkey. J. Comp. Neurol. 2002;442:277. [PubMed: 11774342]
- 99.
- Sandell JH, Peters A. Effects of age on nerve fibers in the rhesus monkey optic nerve. J. Comp. Neurol. 2001;429:541. [PubMed: 11135234]
- 100.
- Peters A, Sethares C, Killiany RJ. Effects of age on the thickness of myelin sheaths in monkey primary visual cortex. J Comp Neurol. 2001;435:241. [PubMed: 11391644]
- 101.
- Makris N, et al. Cortical thinning of the attention and executive function networks in adults with Attention-Deficit/Hyperactivity Disorder. Cerebral Cortex. 2006 in press. [PubMed: 16920883]
- Review Screening for Cognitive Impairment in Older Adults: An Evidence Update for the U.S. Preventive Services Task Force[ 2013]Review Screening for Cognitive Impairment in Older Adults: An Evidence Update for the U.S. Preventive Services Task ForceLin JS, O'Connor E, Rossom RC, Perdue LA, Burda BU, Thompson M, Eckstrom E. 2013 Nov
- Mild Cognitive Impairment.[StatPearls. 2024]Mild Cognitive Impairment.Anand S, Schoo C. StatPearls. 2024 Jan
- Mild cognitive impairment can be distinguished from Alzheimer disease and normal aging for clinical trials.[Arch Neurol. 2004]Mild cognitive impairment can be distinguished from Alzheimer disease and normal aging for clinical trials.Grundman M, Petersen RC, Ferris SH, Thomas RG, Aisen PS, Bennett DA, Foster NL, Jack CR Jr, Galasko DR, Doody R, et al. Arch Neurol. 2004 Jan; 61(1):59-66.
- Review Changes in Cognitive Function in Human Aging.[Brain Aging: Models, Methods, ...]Review Changes in Cognitive Function in Human Aging.Glisky EL. Brain Aging: Models, Methods, and Mechanisms. 2007
- Review Spatial Navigation (Water Maze) Tasks.[Methods of Behavior Analysis i...]Review Spatial Navigation (Water Maze) Tasks.Terry AV Jr. Methods of Behavior Analysis in Neuroscience. 2009
- Successful vs. Unsuccessful Aging in the Rhesus Monkey - Brain AgingSuccessful vs. Unsuccessful Aging in the Rhesus Monkey - Brain Aging
Your browsing activity is empty.
Activity recording is turned off.
See more...